The Impact of Gravity on Life: Does Gravity play a role in evolution?
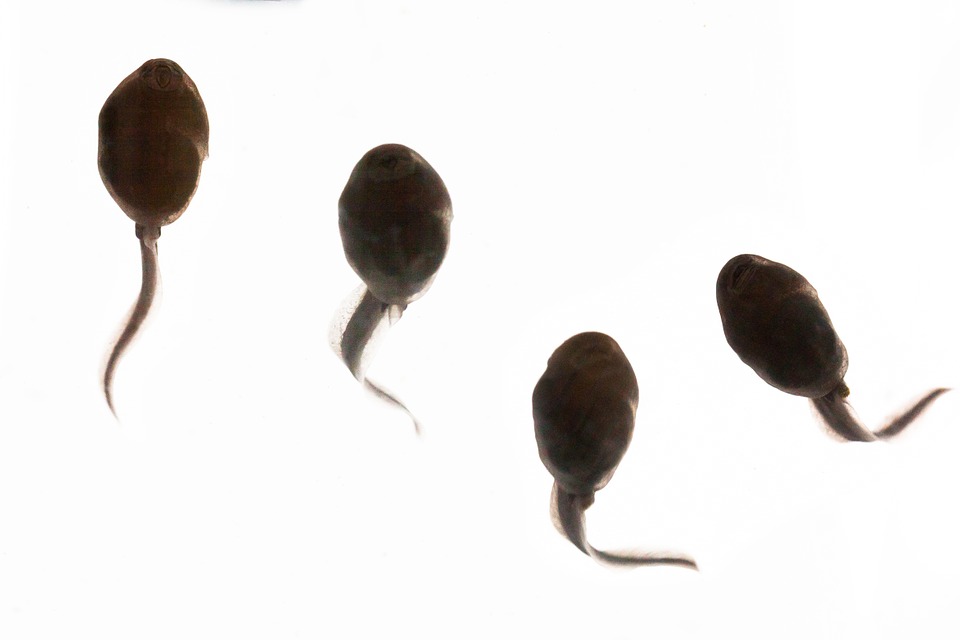
This is the final post in our series The Impact of Gravity on Life, a paper written by Dr. Emily R. Morey-Holton, NASA Ames Research Center, Moffett Field, California. Read the series in its entirety here in blog posts tagged Impact of Gravity on Life.
Gravity affects the environment. Its attractive force gives weight to mass and weight is required for many ecological processes on Earth. Sprinkled throughout this paper are examples and suggestions of the importance of gravity to life, as we know it. Particularly important is the apparent evolutionary development of unique biological structures that amplify the force of gravity and specific gravity sensors that are required for orientation, balance, and movement in a gravity environment. Will these structures and sensors change with gravity levels less than 1G? Only extended time in space with multiple generations will begin to answer this question.
One might predict that plants would grow taller without gravity. Yet, the boundary layers produced by a lack of gravity might concentrate growth-inhibitory or aging factors around the plants, thereby causing them to dwarf; increased gravity might facilitate the dispersal of such factors and actually lead to taller plants. If plants on Earth are fine-tuned to a 1-G environment, then they might not function as well at either increased or decreased gravity levels.
Ecologies, such as algal mats, that stratify by weight on Earth might tend to form as three- dimensional communities without gravity. If the hierarchical structure achieved by stratification is essential for survival or fitness, then the communities would either become extinct or change their fitness leading to evolved characteristics appropriate for the new environment.
Gravity level is important in development of load-bearing structures. The scaling effect of gravity is well known: the % of body mass relegated to structural support is proportional to the size of a land animal (e.g., 20g mouse = ~5%, 70kg human = ~14%, and 7000kg elephant = ~27%). The scaling effect in land animals would likely change in space and could result in a static scale comparable to marine mammals on Earth (~15% of mass as supporting tissues over a wide range of weights).
However, increasing gravity would require altered support structures as scaling up existing structures without any modification in geometry would ultimately lead to failure.
Load-bearing limbs, so important on Earth, are less necessary in space. Human legs not only get in the way during spaceflight but also are involved in the fluid shifts that occur early in flight. Whether legs would disappear over time without gravity (perhaps similar to the extraterrestrial ET) or become more like grasping talons is unknown. Unlike evolution in a decreased gravity environment, higher gravity levels may lead to a different posture and a bipedal stance might become unusual with most species possibly existing as quadrupeds or even hexipeds. Larger species might become extinct at higher gravity levels unless these animals quickly adjust for brain-blood flow and placement of internal organs.
To “fall down” probably requires a certain gravity level and the reflexes related to posture and equilibrium at 1-G are sluggish following spaceflight. Would such reflexes be innervated in species evolving in lower gravity fields? If the reflexes that keep us from falling down in a gravity environment do not develop, then would species evolving in a lower gravity field be able to move when placed in a higher gravity field?
Would the evolutionary response of biological systems be linear, logarithmic, or degraded at gravity levels other than 1-G? Some biological systems (e.g. metabolic rate which is proportional to weight) increase with increased size on Earth. On the other hand, some life systems may have adapted to be maximally efficient at 1-G and degrade with changes in gravity (e.g., body temperature). Life as we know it is extremely adaptable and usually fits form to function during evolution in a hospitable environment. Humans readily adapt to a lower gravity regime aboard spacecraft yet require an extensive “recovery” period when returning to Earth from space voyages suggesting that initial adaptation to a lower gravity environment might be easier than adapting to a higher gravity environment.
A fascinating suggestion that gravity might play a role in evolution comes from snakes. On Earth, snakes have evolved in different environments. For example, tree snakes spend their days crawling up and down trees and exist in an environment where they must cope with gravity. Land snakes spend most of their life in a horizontal position. Sea snakes are neutrally buoyant and spend their life swimming within their habitats. In other words, the orientation of the snake to the direction of the gravity force differs depending upon habitat, without a concomitant alteration in magnitude of gravity. Lillywhite (1988) noticed that the heart of the tree snake was closest to the brain, suggesting that it might be more gravity tolerant than the other snakes as it did not have to carry blood over as great a distance from the heart to the brain. He centrifuged the animals and found that the sea snake had the least gravity tolerance (i.e., fainting with increased gravity), the tree snake had the most, and the land snake was intermediate (Lillywhite et al., 1997).
Changes in heart position, likely related to gravity, most certainly happened over evolutionary, rather than single-generation, time scales. These studies suggest that changes in orientation of a species with respect to the direction of a gravitational force, without an alteration in the magnitude of gravity, may play a role in the evolution of that species on Earth. Gravity may determine the location and size of internal organs such as the heart.
So, what might evolving species at a higher or lower gravity field look like? Form follows function and as function changes, so will form. How much change and what form organisms and ecologies will assume over time in altered gravity is currently unknown. Increasing gravity within a survivable range will probably not cause dramatic differences in evolving Earth-like species while a gravity-free environment will likely produce significant changes in ecologies and species. ET may be a good example of a species evolving at a lower gravity field with the rotund body, duck-like flappers for feet, minimal legs, long thin arm and fingers, and a large head, large eyes, and minimal hair.
To quote ET, “Love your planet”, meaning that you are a product of your physical environment.
We will begin to understand the influence of gravity on evolution of species only after prolonged exposure to different gravity levels. Today, the role of gravity in evolution remains speculative. But one certainly can say that GRAVITY SHAPES LIFE!
ACKNOWLEDGEMENTS
I am most grateful to a number of investigators who have spent many hours discussing various
aspects of their experiments, sharing their unpublished observations with me, and critiquing the manuscript. In particular, I would like to acknowledge Malcolm Cohen, James Kaysen, Cary Mitchell, Lynn Rothschild, and Ken Souza (NASA Ames Research Center), Gail Bingham (Utah State University), Millie Hughes-Fulford (UC-San Francisco and VAMC), David Klaus (University of Colorado/BioServe), Tim Hammond (Tulane University), Tim Jones (University of Missouri/Columbia), Danny Riley (Medical College of Wisconsin), and Kerry Walton (NYU Medical School).
REFERENCES
Alfrey CP, Udden MM, Leach-Huntoon C, Driscoll T, Pickett MH. 1996. Control of red blood cell mass in spaceflight. Journal of Applied Physiology 81:98-104.
Buckey JC, Jr., Gaffney FA, Lane LD, Levine BD, Watenpaugh DE, Wright SJ, Yancy CW, Jr., Meyer DM, Blomquist CG. 1996. Central venous pressure in space. Journal of Applied Physiology 81:19-25.
Brown AH. 1991. From Gravity and the organism to Gravity and the Cell. ASGSB Bull. 4: 7-18.
Fregly MJ, Blatteis CM, eds. 1996. Environmental Physiology: Handbook of Physiology, Section 4 ,
Chapters 29-40. New York: Oxford University Press, 631–970.
Guillen, M. 1995. Five equations that changed the world. Chapter 2: Apples and oranges. New
York:MJF Books, 9-63.
Globus RK, Doty SB, Lull JC, Holmuhamedov E, Humphries MJ, Damsky CH. 1998. Fibronectin
is a survival factor for differentiated osteoblasts. Journal of Cell Science 111:1385-1393. Hammond TG, Lewis FC, Goodwin TJ, Linnehan RM, Wolf DA, Hire KP, Campbell WC, Benes
E, O’Reilly KC, Globus RK, Kaysen JH. 1999. Gene expression in space. Nature Medicine 5:359. Hammond TG, Benes E, O’Reilly KC, Wolf DA, Linnehan RM, Taher A, Kaysen JH, Allen PL,
and Goodwin TJ. 2000. Mechanical culture conditions effect gene expression: gravity-induced
changes on the space shuttle. Physiological Genomics 3:163-173.
Hemmersbach, R, Volkmann, D and Häder, D-P. 1999. Graviorientation in protists and plants.
Journal of Plant Physiology 154: 1-15.
Hughes-Fulford M, Lewis M. 1996. Effects of microgravity on osteoblast growth activation.
Experimental Cell Research 224:103-9.
Ingber DE. 1997. Tensegrity: The Architectural Basis of Cellular Mechanotransduction. Annual
Review of Physiology. 59:575-59.
Ingber DE. 1998. The architecture of life. Scientific American 278:48-57.
Ingber D. 1999. How cells (might) sense microgravity. FASEB Journal 13:S3-S15.
Ingber DE. 2000. The origin of cellular life. BioEssays 22:1160-1170.
Jones, SB, Or, D. 1999. Microgravity effects on water flow and distribution in unsaturated porous
media: analysis of flight experiments. Water Resources Research 35: 929-942.
Jones TA. 1992. Gravity and the ontogeny of animals. The Physiologist 35: S77-79.
Kaysen JH, Campbell WC, Majewski RR, Goda FO, Navar GL, Lewis FC, Goodwin TJ, and
Hammond TG. 1999. Select de novo gene and protein expression during renal epithelial cell
culture in rotating wall vessels is shear stress dependent. Journal of Membrane Biology 168: 77-89. Klaus D, Simski S, Todd P, and Stodieck L. 1997. Investigation of space flight effects on E. Coli and
a proposed model of underlying physical mechanisms. Microbiology 143:449-455.
Klaus DM. Clinostats and Bioreactors. Gravitational and Space Biology Bulletin 14: 55-64. Landis WJ. 1999. Collagen-mineral interaction in vertebrate calcification. Gravitational and Space
Biology Bulletin 12:15-26.
Lillywhite HB 1988. Snakes, blood circulation and gravity. Scientific American. 256:92-98.
Lillywhite HB, Ballard RE, Hargens AR, Rosenberg HI. 1997. Cardiovascular responses of snakes to hypergravity. Gravitational and Space Biology Bulletin. 10:145-152.
Merfeld DM. 1996. Effect of spaceflight on ability to sense and control roll tilt: human
neurovestibular studies on SLS-2. Journal of Applied Physiology 81:50-57.
Merfeld DM, Polutchko KA, Schultz K. 1996. Perceptual responses to linear acceleration after
spaceflight: human neurovestibular studies on SLS-2. Journal of Applied Physiology 81:58-68. Mitchison TJ. 1995. Evolution of a dynamic cytoskeleton. Philosophical Transactions of the Royal
Society of London B Biological Sciences 349:299-304.
Musgrave ME, Kuang A, Porterfield DM. 1997. Plant reproduction in spaceflight environments. Gravitational and Space Biology Bulletin. 10:83-90.
Oman CM, Pouliot CF, Natapoff A. 1996. Horizontal angular VOR changes in orbital and parabolic flight: human neurovestibular studies on SLS-2. Journal of Applied Physiology 81:69-81.
Pace N. 1977. Weightlessness: A matter of gravity. The New England Journal of Medicine 297: 32- 37.
Results of SLS1 and SLS2. 1996. Journal of Applied Physiology 81:3-116.
Ross MD, Tomko, DL. 1998. Effect of gravity on vestibular neural development. Brain Research
Reviews 28:44-51.
Schwuchow J, Sack FD. 1994. Microtubules restrict plastid sedimentation in protonemata of the moss Ceratodon. Cell Motility and Cytoskeleton 29:366-74.
Souza KA, Black SD, Wassersug RJ. 1995. Amphibian development in the virtual absence of gravity. Proceedings of the National Academy of Sciences 92:1975-1978.
Vandenburgh H, Chromiak J, Shansky J, Del Tatto M, Lemaire J. 1999. Space travel directly
induces skeletal muscle atrophy. FASEB Journal 13:1031-1038.
Walton K. 1998. Postnatal development under conditions of simulated weightlessness and space flight.
Brain Research Reviews 28:25-34.
Wayne R, Staves MP, Leopold AC. 1992. The contribution of the extracellular matrix to gravisensing
in characean cells. Journal of Cell Science 101:611-623.
Young LR, Mendoza JC, Groleau N, and Wojcik PW. 1996. Tactile influences on astronaut visual
spatial orientation: human neurovestibular studies on SLS-2. Journal of Applied Physiology 81:44- 49.
WEB ADDRESSES
E. coli: http://www.colorado.edu/ASEN/asen5016/Animation.gif
http://www.colorado.edu/engineering/BioServ
Fundamental forces of nature: http://learn.lincoln.ac.nz/phsc103/lectures/intro/4_forces_of_physics.htm Human kidney cells: http://www.tmc.tulane.edu/astrobiology/microarray
Astrobiology/Life Sciences: http://space.arc.nasa.gov/
http://astrobiology.arc.nasa.gov http://lifesci.arc.nasa.gov